Abstract
In ten families with late-onset ornithine transcarbamylase (OTC) deficiency in male patients, three mutant alleles—R40H, R277W, and Y55D—were identified. In a total of 20 informative parent–offspring pairs, father-to-daughter transmission and mother-to-offspring transmission occurred in five (25%) and 15 (75%), respectively, indicating that paternal transmission contributes substantially to the pool of these mutant alleles. Relative reproductive fitness of males and females carrying the mutant alleles was calculated to be 0.49 and 0.89, respectively. Comparison of the life span of the mutant alleles, estimated on the basis of these fitness values with those associated with classic phenotype (neonatal onset) in which reproductive fitness of male patients was nil, revealed that mutant alleles associated with the late-onset phenotype were eliminated more slowly. This would allow the late-onset phenotype mutant alleles to be retained more frequently in a population than those associated with classic phenotype. Although heterozygous females carrying the late-onset phenotype mutant alleles were generally asymptomatic, one female carrying the R40H allele died after a hyperammonemic episode at the age of 18 years. Such heterozygous females should be alerted to possible hyperammonemic crisis.
Similar content being viewed by others
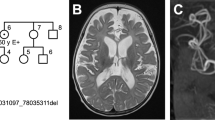
Introduction
Ornithine transcarbamylase (OTC) deficiency (OMIM #311250) is an X-linked disease. Male patients with this enzyme deficiency often develop the disease in the neonatal period or shortly thereafter and generally do not survive infancy (this phenotype is provisionally designated classic in this article). Mutant alleles in the classic phenotype are genetically lethal among male patients but are not necessarily so among females. Among these males, the associated mutant alleles are transmitted, when transmission occurs, exclusively through heterozygous females. In contrast to patients with the classic phenotype, there are known cases of males in whom disease onset is delayed until late childhood (Finkelstein et al. 1990a), adolescence, or even the late 50s (Yoshino et al. 1990) in conditions now collectively termed late-onset OTC deficiency. Nishiyori et al. identified the R40H mutation (1997) and Y55D mutation (1998) in such male patients. In one family carrying the R40H allele, an instance of father-to-daughter transmission as well as mother-to-son transmission of the mutant allele was reported (Nishiyori et al. 1997). Unlike in OTC deficiency with classic phenotype where transmission of the mutant allele occurs exclusively through maternal lineage, in late-onset OTC deficiency, paternal transmission can occur in addition to maternal transmission. The actual proportion of paternal transmission is not yet known.
Reproductive fitness of individuals carrying mutant alleles is a factor that affects the transmission of a mutant allele over generations. Reproductive fitness of heterozygous females who carry mutant OTC alleles is estimated to range between 0.85 (Bonaïte-Pellié et al. 1990) and 0.4 (Tuchman et al. 1995), whereas that of male patients with the classic phenotype is nil. Elucidation of the reproductive fitness of both male and female individuals carrying mutant OTC alleles associated with late-onset OTC deficiency in male patients would contribute to the estimation of mutant allele elimination rate from the gene pool of the general population.
The majority of mutations at the OTC locus are “private” in male patients with classic presentation (Tuchman et al. 1995; Matsuda and Tanase 1997). However, the R40H mutation has been found in discrete families in the Japanese population (Matsuda et al. 1996; Nishiyori et al. 1997; Harada et al. 2006) and in other ethnic groups (Tuchman et al. 1995; Plöchl et al. 1999; Arranz et al. 2007). The R277W mutation was also found in multiple families with different ethnic origins (Finkelstein et al. 1990b; Hata et al. 1991; Matsuura et al. 1993; present series). These observations suggest that these mutant alleles are retained in the general population more frequently than those associated with the classic phenotype. In contrast, the Y55D allele has been found only in two Japanese families to date (Nishiyori et al. 1998; present family).
The study reported here was designed to estimate the proportion mutant allele transmission associated with OTC through paternal lineage and to determine the reproductive fitness of male and female individuals carrying these mutant alleles to derive the elimination rate of such alleles from the gene pool of the general population over generations.
Patients and methods
Patients
Patients 1 through 10 are reported elsewhere (Yoshino et al. 1990; Nishiyori et al. 1997; Harada et al. 2006). In this earlier series, one patient was available from each family, and accordingly, the patient number corresponds to that of the family number. Although later investigations revealed that two pairs of patients were in fact related (patients 1 and 5 and patients 9 and 10), they were enrolled as those from independent families. Among those ten patients, patients 4, 6, and 7 were excluded from the study because either the genotypes of family members other than the patient were not available or the patient had not reproduced by the time of study. Thus, in this study, patients and their family members were enrolled from a total of ten families, which included three previously unreported families. Whereas family numbers and patient numbers used in the original reports are retained in this report to maintain consistency with the previous publications, here we also clearly indicate the family number (F) followed by individual number; for example, patient 2 has the code F2 I-1 (patient 2 in Yoshino et al. 1990). Patients were all males unless otherwise specified. We now present supplementary records for some previously reported patients and their family members and the case reports of previously unreported families.
Patient 2 (F2 I-1, patient 2 in Yoshino et al. 1990) had been well until 46 years of age, when he developed general malaise, anorexia, nausea, vomiting, and abnormal behavior followed by depressed consciousness level. Peak plasma ammonia concentration was 777 μmol/l. He died on the fifth day of illness. OTC activity in the postmortem liver tissue of this patient was <2% of the mean control value. This patient carried the Y55D mutant allele (Nishiyori et al. 1998). One of his maternal uncles had died at age 51 years from what was reported to be fulminant hepatitis.
Patient 4 (F4 III-3), reported elsewhere (Yoshida et al. 1993, patient HO in Nishiyori et al. 1997), had been well until 15 years of age, when he had an episode of fever and headache and took aspirin. Five days after the onset of these symptoms, he had hematemesis, and became irritable and then comatose. Despite courses with sodium benzoate, he succumbed to the disease on the seventh day of illness. Peak plasma ammonia concentration on the sixth day of illness was 1,818 μmol/l. There was marked orotic aciduria. OTC activity in liver tissue was 5.5–10% of the control mean value. Immunoblot analysis revealed that OTC protein was markedly reduced. The apparent Km values of OTC toward l-ornithine and carbamoylphosphate in this patient were similar to those of the wild-type enzyme, and subsequent mutational analysis revealed that this patient had the R40H allele (Nishiyori et al. 1997). Family history was noncontributory.
Patient 6 (F6 III-3, patient AS in Nishiyori et al. 1997) had been well until 10 years of age, when he had headache and vomiting after ingesting a protein-rich meal and experienced general malaise for the next 3 days. On the fifth day of illness, he was admitted due to lethargy and stereotypy. Plasma ammonia concentration on admission was 213 μmol/l. Orotic acid in urine was markedly increased. Continuous venovenous hemodialysis and infusion with sodium benzoate and l-arginine was started, and consciousness was recovered fully within 5 days after admission. OTC activity in liver tissue obtained by biopsy was 10% of the normal control mean value. His subsequent clinical course has been uneventful. Family history was unremarkable.
Patient 7 (F7 II-1, patient HE in Nishiyori et al. 1997) had experienced some episodes of vomiting a couple of times per year in his childhood. At age 17 years, he was admitted to hospital because of consciousness disturbance that developed following episodes of nausea lasting for 2 weeks. Plasma ammonia concentration on admission was 228 μmol/l. The patient received lactulose and aminoglycoside, followed by ammonia adsorption therapy. By the sixth day of hospitalization, his consciousness level was alert. Measurement of OTC activity in the liver, carried out elsewhere, revealed it to be 7% of the normal control mean. He has been well since discharge. Family history was noncontributory.
The younger sister (F3 II-4) of patient 3 (F3 II-3, Yoshino et al. 1990) began to develop episodes of vomiting after ingesting a protein-rich meal at age 13 years and died from hyperammonemic coma at age 18 years. The daughter (F8 II-1) of patient 8 (F8 I-1), aged 17 years at the time her father died, had motor paresis and mental retardation of unknown etiology. There was no other symptomatic heterozygous female other than these two girls. The following patients have not been reported previously.
Patient 11 (F11 II-1) had been well until 10 years of age, except for short stature, when he first developed nausea, vomiting, and agitation after he began ingesting cow’s milk 1 month prior to the disease onset. He was found to have hyperammonemia and orotic aciduria (1,910 μmol/mmol creatinine, normal fasting maximum <1.5). OTC activity in the liver was 2.0% of the control mean. He has been placed on a regular diet and takes medication with sodium benzoate and l-arginine. The patient is physically and intellectually normal at the age of 23 years. Patient 12 (F11 II-3), the younger brother of patient 11, had repeated episodes of vomiting after meals between the ages of 2 and 3 years, but such episodes spontaneously resolved. He was examined at age 4 years and found to have decreased liver OTC activity (2% of control mean). This patient has a similar treatment regimen to that of his brother and is physically and intellectually normal at the age of 16 years. Mutational analysis of the OTC gene revealed both patients had the R277W mutation.
Patient 13 (F12 II-1) had been normal until 3 years of age when he developed vomiting and lethargy after a brief febrile episode. The boy was transferred to hospital, where hyperammonemia and orotic aciduria were found. Despite repeated courses of hemodialysis, the patient remained comatose, and cardiac arrest ensued 30 days later. Urgent mutational analysis was made with his peripheral blood to confirm the diagnosis because his mother was in the third trimester of pregnancy when patient 13 first developed signs of hyperammonemia. The analysis revealed patient 13 carried the Y55D mutant allele. Patient 14 (F12 II-2) was the younger brother of patient 13. The boy was born after 38 weeks and 5 days of gestation, and birth weight was 3,020 g. His perinatal course was uneventful, although the peak plasma ammonia concentration was 110 μmol/l. Mutational analysis after birth revealed the Y55D allele. Patient 14 has been under close medical observation while on a regular diet without any medication, with his parents’ consent after thorough discussion with them. His plasma ammonia concentration has never exceeded 35 μmol/l on this regimen, and the patient has achieved normal growth and developmental milestones at the age of 3 years.
Patient 15 (F13 IV-2) was reported elsewhere (Yasutake et al. 2007). In brief, the patient had been in good physical and mental condition until 16 years of age, when he began to vomit and complain of fatigue after physical exercise. On the eighth day of illness, he became agitated, and his consciousness level decreased, so he was admitted to hospital. Laboratory studies on admission revealed hyperammonemia (988 μmol/l), and increased plasma glutamine concentration, and orotic aciduria. A son of a younger sister of the maternal grandfather of patient 15 had died at age 14 years of what was reported to be OTC deficiency.
These families were independent from each other in terms of any factors that may affect fecundity, although they shared genetic background. Family charts of these patients are available as electronic supplementary material.
Determination of genotype
Genotype was determined by one of the following methods or a combination thereof: assay of OTC activity in the liver (E), OTC gene analysis including DNA sequencing or restriction fragment-length polymorphism (RFLP) (G), biochemical analysis (B, presence of hyperammonemia and orotic aciduria), or by the pedigree analysis (P). Criteria for genotype determination are indicated by these abbreviations in the parentheses in Table 1. Mutational analysis of the OTC gene was performed in each proband.
Mutational analysis of OTC gene and biochemical analyses
DNA was isolated from peripheral blood or liver tissue obtained by autopsy by standard methods. Polymerase chain reaction (PCR) amplification of OTC gene fragments was conducted, as previously described (Matsuura et al. 1993), and PCR products were directly sequenced or subjected to analysis by RFLP generated by NlaIII as described elsewhere (Nishiyori et al. 1997). Liver enzyme assay and determination of orotic acid were carried out by conventional methods (Yoshino et al. 1990).
Reproductive fitness of individuals carrying OTC mutant alleles, definition of reproductive age, and elimination patterns of mutant alleles
Reproductive fitness of a genotype is defined as efficiency in producing offspring, and it is in practice represented as relative fitness; the proportion of offspring that survive to reproductive age related to that of the optimum—most fecund—genotype (Vogel and Motulsky 1997a). In the study reported here, individuals who carried a mutant allele and had offspring that had reached reproductive age were enrolled. The number of offspring of each carrier of a mutant allele was related to the total period fertility rate (Vital Statistics of Japan 1900–2001) for a respective year when the individual first reproduced, because total period fertility rate varied significantly over the years in Japan. Number of offspring was counted 0 when an individual had a spouse but had no offspring who had survived to reproductive age in a mentally and physically intact condition. The lower limit of reproductive age was defined as 15 years for females and 18 years for males, the ages when individuals contribute to >0.1% of the yearly birth number (1.14 × 106) in Japan (Kühnert and Nieschlag 2004). The values of relative fitness of males (w m) and females (w f) were applied to estimate the elimination rate of mutant OTC alleles.
Ethical considerations
Patients or their authorized surrogates provided informed consent to participate in this study. The study protocol was approved by the institutional review board of Kurume University School of Medicine and conforms to the provisions of the Declaration of Helsinki in 1995 (as revised in Edinburgh 2000).
Results
Mutational analysis in probands
The R40H (g.119G > A, CGT > CAT) mutation was found in patients 1, 3–10, and 13 (Tables 1, 2). The Y55D (g.163T > G, TAT > GAT) mutation was identified in patient 2 of family 2 and patients 13 and 14 of family 12. The siblings of family 11 (patients 11 and 12) were determined to carry the R277W (g.829C > T, CGG > TGG) mutation.
Comparison of mutant allele transmission via paternal and maternal lineages
In seven families carrying the R40H mutation, 15 informative pairs of a parent and offspring were available for analysis (Table 1). There were three pairs available for study in two families carrying the Y55D mutation, and two pairs in one family carrying the R277W mutation (Table 1). In the families carrying the R40H allele, there were four instances of mother-to-daughter transmission, seven instances of mother-to-son transmission, and four instances of father-to-daughter transmission. In two families carrying Y55D, there were two instances of mother-to-son transmission and one instance of father-to-daughter transmission. In the family carrying the R277W mutation, there were two instances of mother-to-son transmission. Thus, there occurred overall a total of five instances of father-to-daughter transmission and a total of 15 instances of mother-to-offspring transmission.
Relative fitness of carriers of mutant alleles
Relative fitness of males (w m) and of females (w f) carrying the mutant alleles was found to be 0.49 and 0.89, respectively, as all family members harboring each one of the three mutant alleles were combined (Table 2).
Elimination rate of mutant alleles associated with late-onset phenotype
In this study, estimation was made concerning how reproductive fitness of individuals carrying the three mutant OTC alleles affects their transmission over generations. The primary gender ratio of humans, that is, the ratio of fertilization of the ovum with an X chromosome bearing spermatozoon or Y chromosome bearing spermatozoon, is estimated to be not far from 1:1 (male:female), although it is somewhat affected by several factors, including the interval between sexual intercourse and ovulation (Vogel and Motulsky 1997b). Let the probability of transmission of a sex chromosome from parent to offspring be p, and that of the other sex chromosome be q (q = 1 − p), relative fitness for males be w m, and that of females be w f, and the probabilities of transmitting an X chromosome carrying the mutant allele (X) from a heterozygous mother to a daughter or a son are given as follows:
The probability of transmission of the mutant allele from a hemizygous father to his daughter is given as follows:
While the mutant allele is not transmitted from a hemizygous male to his son, then,
Thus, the ratio of probability of transmission of the mutant allele through paternal lineage to that through maternal lineage is:
since p + q = 1.
Given that there is no selection bias between an X chromosome carrying the mutant allele and either X chromosome carrying the wild-type allele or Y chromosome on transmission to an offspring, p = q = 0.5. Then, the above ratio is simplified to be w m:w f. This result means that the ratio of paternal transmission to maternal transmission is determined solely by respective relative reproductive fitness.
The probability that a mutant allele is transmitted from an individual who carries the allele (F1) to its offspring in a given generation (Fi) can be given as follows:
Likewise,
Thus, the probability that the mutant allele is transmitted to offspring in a given generation (Fi) from a heterozygous female can be given as follows:
The probability that the mutant allele is transmitted to offspring in a given generation (Fi) from a hemizygous male can be given as follows:
Solving Eqs. 1 and 2 recursively with w m = 0.49, w f = 0.89, and p = q = 0.5, profiles of these probabilities over generations are plotted in Fig. 1, indicating that the transmission probabilities decrease gradually over generations.
Selection patterns of mutant ornithine transcarbamylase (OTC) alleles associated with late-onset phenotype and classic phenotype over generations. Probability of transmission of mutant alleles associated with late-onset phenotype from heterozygous female (filled diamond), hemizygous male (filled square), and the sum of both (filled triangle) to their offspring, and that from heterozygous female carrying classic phenotype mutant alleles (filled circle)
Effect of reproductive fitness on transmission of a mutant allele associated with classic phenotype
Fitness of male patients with classic phenotype is nil; consequently, a mutant allele is transmitted exclusively through maternal lineage. In this situation, the above-mentioned calculation requires some modification.
where,
Hence
Furthermore, since
the probability that offspring in a given generation (Fi) inherit the mutant allele is
When a relative fitness of heterozygous female of 0.4 (Tuchman et al. 1995) is applied to the above equation, it gives 0.4 × 0.5(0.4 × 0.5 × 0.5)(i−2) = 0.2(0.1)(i−2), which is also plotted in Fig. 1.
Discussion
It was demonstrated in one family that father-to-daughter transmission of the OTC R40H allele could occur (Nishiyori et al. 1997). However, to what extent father-to-daughter transmission contributes to the pool of such mutant alleles in the population is not known. In the study reported here, father-to-daughter transmission of a mutant allele was found in 25% (5 of 20) of informative parent–offspring pairs. Thus, the contribution of paternal transmission to the gene pool cannot be disregarded. Mother-to-daughter transmission was observed in 20% (4 of 20) and mother-to-son transmission in 55% (11 of 20). This difference between transmission to sons and to daughters is most likely due to, firstly, bias with regard to the ascertainment of heterozygotes in the daughters for whom gene analysis was not available and, secondly, the possible presence of heterozygotes among daughters who were not examined.
The relative reproductive fitness of hemizygous males and heterozygous females carrying mutant alleles associated with the late-onset phenotype was found to be 0.49 and 0.89, respectively. Thus, these mutant alleles are eliminated over generations (Eqs. 1, 2, Fig. 1; raw data derived from these equations is available as electronic supplementary material). According to these equations, provided that both maternal and paternal transmissions are combined, the probability that a mutant allele in a given generation can be transmitted to its offspring, for example, two generations downstream (generation 3) is 0.208. However, in the model for mutant alleles associated with the classic phenotype (Eq. 3), in the case that reproductive fitness of males is nil and that of females is 0.4 (Tuchman et al. 1995), the probability in the corresponding generation is 0.02. These results indicate that the mutant alleles associated with the late-onset phenotype are eliminated exponentially slower than those associated with the classic phenotype. The phenotypic presentation in the small numbers of individuals carrying these mutant alleles could be diverse. Accordingly, the elimination rate may be variable in each mutant allele, although this would be impossible to determine in reality.
The values of relative reproductive fitness of individuals carrying alleles with late-onset phenotype derived here are obviously provisional. Differences in the elimination rate between mutant alleles associated with late-onset phenotype and classic phenotype would be further exaggerated depending on the sociomedical status of the patient, because late-onset phenotype is amenable to adequate medical intervention, whereas in the classic phenotype, prognosis is poor regardless of medical intervention.
Mutations of OTC alleles in patients with classic phenotype are diverse in the majority of families (McCullough et al. 2000). In contrast, certain mutations were repeatedly found in families in the series reported here that are reportedly unrelated, although all the R40H mutant alleles in the series were found in families living in an area with a radius of 120 km, suggesting a possible common ancestral origin. In addition, this mutant allele has occurred recurrently (Tuchman et al. 1995; Plöchl et al. 1999; Arranz et al. 2007; Pinner et al. personal communication). Thus, R40H alleles may be maintained mostly by transmission from ancestors to their offspring, with occasional addition by de novo mutation.
The R277W allele and Y55D allele may have been retained in the population studied at a certain frequency because the vast majority of individuals carrying those alleles, particularly heterozygous females, were asymptomatic or oligosymptomatic. The R277W mutation has been recurrently observed in Hispanic/Caucasian populations (Finkelstein et al. 1990b; McCullough et al. 2000), as well as in Japanese (Hata et al. 1991; Matsuura et al. 1993; present patients). In contrast, the Y55D mutant allele was previously reported in only one patient who first developed hyperammonemic crisis and died at the age of 48 years (Yoshino et al. 1990; Nishiyori et al. 1997). This single nucleotide change (g.163T > G) is the least frequent among the transversions and transitions (Tuchman et al. 1996) and is not in the CpG nucleotide in the OTC gene. The occurrence of the identical mutation in two discrete families is therefore probably the product of founder-effect mutations.
Symptomatic heterozygous females almost exclusively carry mutations associated with the classic phenotype (McCullough et al. 2000), whereas heterozygous females in the series reported here remained generally asymptomatic. This is very likely due to the less deleterious nature of these mutant alleles. However, to our knowledge, two heterozygous females who carried the R40H allele developed hyperammonemic crisis and died (the sister of patient 3 in this series and one in Pinner et al. personal communication). Extremely skewed X inactivation in favor of the mutant allele in such females would result in a decreased capacity of ammonia detoxification, as in hemizygous male patients. The experience reported here indicates that females who carry the R40H mutation should be alerted to possible hyperammonemic crisis.
References
Arranz JA, Riudor E, Marco-Marín C, Rubio V (2007) Estimation of the total number of disease-causing mutations in ornithine transcarbamylase (OTC) deficiency. Value of the OTC structure in predicting a mutation pathogenic potential. J Inher Metab Dis 30:217–226. doi:https://doi.org/10.1007/s10545-007-0429-x
Bonaïti-Pellié C, Pelet A, Ogier H, Nelson J-R, Largillière C, Bertlelot J, Saudubray J-M, Munnich A (1990) A possible sex difference in mutation rates in ornithine transcarbamylase deficiency. Hum Genet 84:163–166
Finkelstein JE, Hauser ER, Leonard CO, Brusilow SW (1990a) Late-onset ornithine transcarbamylase deficiency in male patients. J Pediatr 117:897–902
Finkelstein JE, Francomano CA, Brusilow SW, Traystman MD (1990b) Use of denaturing gradient gel electrophoresis for detection of mutation and prospective diagnosis in late onset ornithine transcarbamylase deficiency. Genomics 7:167–172
Harada E, Nishiyori A, Tokunaga Y, Watanabe Y, Kuriya N, Kumashiro R, Kuno T, Kuromaru R, Hirose S, Ichikawa K, Yoshino M (2006) Late-onset ornithine transcarbamylase deficiency in male patients: prognostic factors and characteristics of plasma amino acid profile. Pediatr Int 48:105–111. doi:https://doi.org/10.1111/j.1442-200X.2006.02181.x
Hata A, Matsuura T, Setoyama C, Shimada K, Yokoi T, Akaboshi I, Matsuda I (1991) A novel missense mutation in exon 8 of the ornithine transcarbamylase gene in two unrelated male patients with mild ornithine transcarbamylase deficiency. Hum Genet 87:28–32
Kühnert B, Nieschlag E (2004) Reproductive functions of ageing male. Hum Reprod Update 10:327–339. doi:https://doi.org/10.1093/humupd/dmh030
Matsuda I, Matsuura T, Nishiyori A, Komaki S, Hoshide R, Matsumoto T, Funakoshi M, Endo F, Hata A, Shimadzu M, Yoshino M (1996) Phenotypic variability in male patients carrying the mutant ornithine transcarbamylase (OTC) allele Arg40His, ranging from a child with an unfavourable prognosis to an asymptomatic older adult. J Med Genet 33:645–648
Matsuda I, Tanase S (1997) The ornithine transcarbamylase (OTC) gene: mutations in 50 Japanese families with OTC deficiency. Am J Med Genet 71:378–383
Matsuura T, Hoshide R, Setoyama C, Shimada K, Hase Y, Yanagawa T, Kajita M, Matsuda I (1993) For novel gene mutations in five Japanese male patients with neonatal or late onset OTC deficiency: application of PCR-single strand conformation polymorphisms for all exons and adjacent introns. Hum Genet 92:49–56
McCullough BA, Yudkoff M, Batshaw ML, Wilson JM, Raper SE, Tuchman M (2000) Genotype spectrum of ornithine transcarbamylase deficiency: correlation with the clinical and biochemical phenotype. Am J Med Genet 93:313–319
Nishiyori A, Yoshino M, Kato H, Matsuura T, Hoshide R, Matsuda I, Kuno T, Miyazaki S, Hirose S, Kuromaru R, Mori M (1997) The R40H mutation in a late onset type of human ornithine transcarbamylase deficiency in male patients. Hum Genet 99:171–176
Nishiyori A, Yoshino M, Tananari Y, Kato H, Matsuura T, Hoshide R, Matsuda I, Kato H (1998) Y55D mutation in ornithine transcarbamylase associated with late-onset hyperammonemia in a male. Hum Mutat (Suppl 1)S131–S133
Plöchl W, Spiss CK, Plöchl E (1999) Death after transplantation of a liver from a donor with unrecognized ornithine transcarbamylase deficiency. N Engl J Med 341:921
Tuchman M, Matsuda I, Munnich A, Malcolm S, Strautnieks S (1995) Proportions of spontaneous mutations in males and females with ornithine transcarbamylase deficiency. Am J Med Genet 55:67–70
Tuchman M, Plante RJ, García-Pérez MA, Rubio V (1996) Relative frequency of mutations causing ornithine transcarbamylase deficiency in 78 families. Hum Genet 97:274–276
Vital Statistics of Japan (1900–2001) Statistics and Information Department, Ministry of Health. Labour and Welfare of Japan
Vogel F, Motulsky AG (1997a) Human genetics—problems and approaches, 3rd edn. Springer, Berlin, pp 508–516
Vogel F, Motulsky AG (1997b) Human genetics—problems and approaches, 3rd edn. Springer, Berlin, p 137
Yasutake T, Nagatomo D, Mizuta T, Eguchi Y, Kumagai T, Ario K, Ozaki I, Kuno T, Fujimoto K (2007) Gekishougata de hasshou si, fukou na tenki wo totta chihatsu-gata orunichintoransukarubamira-ze kessonshou no ichi-rei [Late-onset type ornithine transcarbamylase deficiency (OTCD) with fulminant onset following a fatal prognosis, author’s translation]. Jpn J Gastroenterol (in press)
Yoshida I, Yoshino M, Watanabe J, Yamashita F (1993) Sudden onset of ornithine carbamoyltransferase after aspirin ingestion. J Inher Metab Dis 16:917
Yoshino M, Nishiyori J, Yamashita F, Kumashiro R, Abe H, Tanikawa K, Ohno T, Nakao K, Kaku N, Fukushima H, Kubota K (1990) Ornithine transcarbamylase deficiency in male adolescence and adulthood. Enzyme 43:160–168
Acknowledgment
This work was supported partly by grants-for-aid for scientific research from the Ministry of Education, Culture, Sports, Science and Technology of Japan, and by a grant from the Research Center of Innovative Cancer Therapy of the 21st Century COE Program for Medical Science, Kurume University.
Author information
Authors and Affiliations
Corresponding author
Electronic supplementary material
Rights and permissions
About this article
Cite this article
Numata, S., Harada, E., Maeno, Y. et al. Paternal transmission and slow elimination of mutant alleles associated with late-onset ornithine transcarbamylase deficiency in male patients. J Hum Genet 53, 10–17 (2008). https://doi.org/10.1007/s10038-007-0212-8
Received:
Accepted:
Published:
Issue Date:
DOI: https://doi.org/10.1007/s10038-007-0212-8
Keywords
This article is cited by
-
Father-to-daughter transmission in late-onset OTC deficiency: an underestimated mechanism of inheritance of an X-linked disease
Orphanet Journal of Rare Diseases (2024)
-
Mutant alleles associated with late-onset ornithine transcarbamylase deficiency in male patients have recurrently arisen and have been retained in some populations
Journal of Human Genetics (2010)