Abstract
We report reaction temperature sensing (RTS)-based control to fundamentally enhance Li-ion battery safety. RTS placed at the electrochemical interface inside a Li-ion cell is shown to detect temperature rise much faster and more accurately than external measurement of cell surface temperature. We demonstrate, for the first time, that RTS-based control shuts down a dangerous short-circuit event 3 times earlier than surface temperature- based control and prevents cell overheating by 50 °C and the resultant cell damage.
Similar content being viewed by others
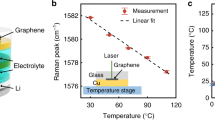
Introduction
Safety of large-scale Li-ion batteries has become a critical issue, propelled by ever-increasing applications in electric vehicles (EVs)1,2,3,4,5. Recent high-profile events involving electric cars and airplanes that employ Li-ion batteries as main or auxiliary power sources lend further urgency to improved Li-ion battery safety6,7. When safety is breached, Li-ion batteries may experience thermal runaway causing significant equipment or personnel damage3,8,9.
While Li-ion materials and chemistry have been well understood for over two decades, the engineering science of large Li-ion batteries remains elusive, as evident from the recent battery fire accident on Boeing Dreamliner 787. Early detection and protection technologies are essential elements of large Li-ion battery systems for vehicle, aircraft and grid energy storage applications9,10. Ability to measure the temperature precisely at the electrochemical reaction interface inside a cell provides sensitive monitoring of the health and safety states of the Li-ion cell9,11,12,13,14,15. The close correlation of the reaction temperature to battery internal state offers an excellent means for early detection of potential faults allowing timely intervention.
Some previous studies16,17,18,19,20 and our recent study21 on Li-ion battery internal temperature show that the internal temperature at the reaction area responds faster than surface temperature, especially when heat is generated rapidly such as during short circuit and overcharge16,17,18.We hypothesize that early detection and prevention of Li-ion battery safety failure can be realized through internal reaction temperature sensing (RTS) and a control algorithm based on RTS. Different from existing approaches that monitor voltage, current, or other sophisticated signal processing methods10,22,23,24,25,26,27,28 of individual cells or modules for early signs of abnormality, RTS directly monitors the temperature at the reaction surface, a critical parameter determining safety status of the Li-ion battery.
In this study, a novel RTS-based control strategy is developed to improve Li-ion battery safety. Different from previous studies16,17,18 in which internal temperature is just measured and compared with surface temperature during abuse testing, this study focuses more on using the faster response of internal reaction temperature for early detection and prevention of battery safety failure. This RTS-based control strategy can detect abnormal temperature rise inside Li-ion battery cells much faster and much more accurately than conventional surface temperature based control, thereby allowing for earlier intervention to prevent damage from safety breach. We demonstrate the effectiveness of this technique by fabricating Li-ion battery cells with internal reaction temperature sensors and testing them under a short-circuit condition, a common safety failure scenario for Li-ion batteries. By terminating the shorting when cell internal reaction temperature or surface temperature reaches a prescribed threshold, say 80 °C, we show that RTS-based control provides very effective early detection and prevention of Li-ion battery safety failures.
Results
Shorting test with RTS-based control
To demonstrate the effectiveness of RTS-based control for Li-ion battery safety, experimental Li-ion cell with embedded RTS sensor is fabricated in the Battery Manufacturing Lab at The Pennsylvania State University. Figure 1(a) shows the schematic of a cylindrical cell with an embedded RTS. A similar temperature sensor is also placed on the cell outer surface for comparison between RTS and external surface temperature (Tsurf). The experimental cell in this study has a nominal capacity of 1.6 Ah. It is fully charged and then short circuited using a specially designed experimental system that can terminate shorting automatically when RTS reaches threshold. A schematic of the experimental system is shown in Fig. 1(b). Details of fabricating RTS cells and developing the experimental system are described in the Methods section.
Figure 2 shows the variation of cell voltage, current, reaction temperature (RTS) and surface temperature (Tsurf) of the cell during shorting test with RTS-based control. The threshold temperature is 80 °C. This threshold is chosen because decomposition of solid electrolyte interface (SEI) and electrolyte can occur at around 80 °C29,30. It can be seen that as shorting begins, the voltage drops abruptly to around 0.7 V and the current reaches as high as 63 A, approximately 40 times that during 1C discharge. Such voltage and current behaviors are typical for Li-ion battery cells during short circuit31. Very low cell voltage and very high current suggests high rate of heat generation. While both reaction temperature and surface temperature increase dramatically, reaction temperature increases much more rapidly than surface temperature. At 5.5 s the reaction temperature reaches 80 °C and the shorting is terminated successfully by RTS-based controller. Then the reaction temperature reaches maximum value immediately and begins decreasing. In comparison, the surface temperature is only 50 °C at shorting termination and keeps increasing due to heat transfer from the cell interior. It reaches maximum value of 53 °C at 25 s, much lower and much later than the reaction temperature. Comparison with reaction temperature shows that surface temperature responds much more slowly and fails to detect the highest temperature a Li-ion battery cell could experience during abnormal operation. This successful termination of short circuit suggests that RTS-based control is effective in early detection and prevention of safety failure of Li-ion battery cells.
Variation of cell voltage, current, reaction temperature (RTS) and surface temperature (Tsurf) of experimental Li-ion cell during shorting test with RTS-based control (Tthreshhold=80 °C).
At 5.5 s the reaction temperature reaches 80 °C and the shorting is terminated successfully. In comparison, the surface temperature is only 50 °C at shorting termination.
Shorting test with surface temperature- based control
To further demonstrate the advantage of RTS-based control over surface temperature- based control, the cell is short circuited one more time with termination of shorting based on the surface temperature sensing. The threshold temperature is still kept at 80 °C. Figure 3 shows variation of cell voltage, current, reaction temperature and surface temperature (Tsurf) of the cell during test with surface temperature- based control. It can be seen that cell voltage, current, reaction temperature and surface temperature behave similarly to those in the previous case as shorting starts. The shorting is terminated successfully when the surface temperature reaches 80 °C, but lasts 16 s before termination. This is 3 times longer than demonstrated with RTS-based control. Moreover, the internal reaction temperature has reached 129 °C when the shorting stops, which is ~50 °C higher than the threshold safety temperature and close to the melting temperature of the separator (~135 °C for polyethylene based separator)31,32, suggesting possible damage to the cell. Although the internal reaction temperature begins decreasing immediately after termination of shorting, it stays above threshold temperature of 80 °C for over 150 s. The surface temperature keeps increasing until reaching maximum value of 87.6 °C at 38.5 s, more than 20 s later than shorting termination. In comparison, the internal reaction temperature reaches maximum value at essentially the same time as shorting is terminated. The maximum surface temperature underestimates the maximum temperature inside the cell by more than 40 °C. Comparison of the internal reaction temperature and surface temperature shows again that the former responds much faster than the latter and provides a much more accurate monitor of highest temperature inside Li-ion battery cells under extreme conditions.
Variation of cell voltage, current, reaction temperature (RTS) and surface temperature (Tsurf) of experimental Li-ion cell during shorting test with surface temperature- based control (Tthreshhold=80 °C).
The shorting is terminated when surface temperature reaches 80 °C, but it lasts 16.0 s, ~3 times of that with RTS-based control. The maximum reaction temperature reaches ~50 °C higher than the threshold temperature.
Comparison of performance after shorting tests
The results above show that cell temperature reaches 129 °C and stays higher than 80 °C for over 150 seconds with surface temperature- based control. Therefore, damage could have been caused to the Li-ion battery cell. To verify if the shorting cause damage and whether RTS-based control can avoid such damage, the Li-ion cell is tested after each shorting test. It is firstly fully charged and then fully discharged at 1C (1.6 A). The results are shown in Fig. 4. The performance before shorting test is also presented for comparison. It can be seen that there is no decrease of performance after shorting with RTS-based control. In comparison, cell performance shows obvious decrease (3%) after shorting with surface temperature- based control. The results confirm that RTS-based control can indeed avoid overheating and consequent damages.
Discussion
The setting of termination temperature at 80 °C in above tests is conservative. When the termination temperature is set at higher temperature, much more serious damage could ensue with surface temperature- based control. We tested this hypothesis by further setting the termination temperature at 100 °C (See Supplement Figs 1, 2, 3). The highest internal temperature reached 137 °C and an additional 12% decrease of performance was observed after the shorting experiment with surface temperature- based control. In contrast, RTS-based control effectively detects abnormal temperature rise and prevents damages from overheating in the case of termination temperature at 100 °C.
Early detection and protection technologies are an essential part of large Li-ion batteries and their systems for vehicle, aircraft and grid energy storage applications. In this work we have developed a technique based on internal reaction temperature sensing (RTS) to detect abnormal temperature rise early and intervene early so as to prevent battery failure and catastrophic accident. Using a self-built 18650 Li-ion cell with capacity of 1.6 Ah, we showed that RTS-based control can detect abnormal temperature rise during shorting ~3 times earlier than surface temperature- based control. This technique prevents overheating of the Li-ion cell by ~50 °C and avoids subsequent damages from that overheating. The experimental results show that RTS-based control holds great promise for early detection and prevention of Li-ion battery safety breach. Further research based on this technique, including its application in other safety failure scenarios and in larger Li-ion batteries and packs, as well as development of more sophisticated sensors and control algorithms, is warranted.
Moreover, when cells with higher energy density experience shorting, internal temperature would be higher and much more serious damage could occur. Great challenges lie ahead to ensure safety of high energy density batteries, such as Li-S and Li-O2 batteries33,34, which are required for future electric transportation with much improved cruise range. The results in this study show that the RTS-based control will be an effective strategy for early detection of abnormal thermal behaviors and prevention of safety failures of future high energy density batteries.
Methods
Design and fabrication of Li-ion cells with RTS
As schematically shown in Fig. 1(a), cylindrical Li-ion battery cells (format 18650, diameter of 18 mm and height of 65 mm) are designed with embedded micro reaction temperature sensor (RTS) for internal reaction temperature diagnosis. The micro temperature sensor is located at innermost end of the reaction area of a cylindrical Li-ion cell where temperature is highest along the radial direction21. Comparing with fabricating conventional cylindrical Li-ion cells35, there are four additional steps in fabricating cells with RTS: (1) Coating micro temperature sensor with parylene for anti-corrosion in operating Li-ion cell condition; (2) Embedding the sensor at the reaction interface between negative electrode and separator near the innermost end of jelly roll during winding process; (3) Inserting the jelly roll with embedded sensor in a stainless steel can with a pre-drilled micro hole on the wall for extension of senor out of the can; (4) Sealing the micro hole on the wall of the can with epoxy before filling electrolyte and crimping of the cell.
Micro temperature sensors used in this study are T type micro thermocouples (600T, RTD Company, USA) with wire diameter of 80 um and an additional 10 um insulation. The micro thermocouples have no insulation on the measuring tip as received. A 10 μm layer of parylene is coated on the measuring tip using a special parylene evaporator at Penn State Materials Research Institute Nanofabrication Lab. With insulation, thickness of the sensor measuring tip is 100 um, identical to that of the wire. Another micro temperature sensor is placed on the outer surface of the cell to monitor surface temperature (Tsurf) and compare with RTS.
Experimental cells are made in the Battery Manufacturing Lab at The Pennsylvania State University, using LiNi1/3Co1/3Mn1/3O2 (NCM) and graphite as positive and negative electrode materials, respectively. Thicknesses of the positive electrode and negative electrode are 150 um and 140 um respectively, including current collecting collector and coating on both sides. The positive current collector is aluminum foil of 15 um and negative current collector is copper foil of 10 um. The separator is Celgard® 2320 PP/PE/PP trilayer membrane with thickness of 20 um. The electrolyte is 1.2 M LiPF6 in EC:EMC:DMC (20:20:60 v%).
Development of experimental system to trigger and terminate short circuit
To verify the effectiveness of the RTS, we develop an experimental system that can trigger and terminate short circuit of an experimental Li-ion cell. Figure 1(b) shows the experimental system schematically. A shunt resistor (0.15 mΩ, ±0.5%, OHMITE, USA) is used to measure shorting current of the cell. The total external shorting resistance is 10 mΩ, including all resistance outside the cell, which is measured by a low resistance meter (3560, Hioki, Japan). A temperature controller (CN8201, OMEGA Engineering, USA) and a contactor (LEV200, Tyco Electronics, USA) are used for starting and terminating short circuit. A multi-channel data acquisition unit (34970A, Agilent Technologies, USA) is used to record the internal reaction temperature, surface temperature, current and voltage of the cell during test every 0.5 s. A battery tester (BT2000, Arbin Instruments, USA) is used to fully charge the cell before shorting test and to characterize cell performance after shorting test. The shorting test is carried out in a safety chamber which provides natural convection cooling condition.
Cell charging protocol
The cell is fully charged using Constant Current-Constant Voltage (CC-CV) protocol (0.8 A, 4.2 V max, 0.032 A cut-off) at room temperature (25 ± 1 °C). Then the cell is rested for at least one hour, allowing open circuit voltage (OCV) and cell temperature to reach equilibrium, before shorting test or performance characterization after shorting test.
Additional Information
How to cite this article: Zhang, G. et al. Reaction temperature sensing (RTS)-based control for Li-ion battery safety. Sci. Rep. 5, 18237; doi: 10.1038/srep18237 (2015).
References
Armand, M. & Tarascon, J. M. Building better batteries. Nature 451, 652–657, 10.1038/451652a (2008).
Whittingham, M. S. Lithium batteries and cathode materials. Chem. Rev. 104, 4271–4301, 10.1021/cr020731c (2004).
Doughty, D. & Roth, E. P. A general discussion of Li ion battery safety. ECS Interface Summer, 37-44 (2012) Available at: http://www.electrochem.org/dl/interface/sum/sum12/sum12_p037_044.pdf (Accessed: 23rd December 2014).
Goodenough, J. B. & Park, K.-S. The Li-ion rechargeable battery: a perspective. J. Am. Chem. Soc. 135, 1167–1176, 10.1021/ja3091438 (2013).
Rahn, C. D. & Wang, C. Y. Battery management systems in Battery systems engineering, Ch.8, 224–228, 10.1002/9781118517048.ch8 (John Wiley & Sons Ltd, 2013).
Smith, B. Chevrolet Volt battery incident overview report. Technical Report DOT HS 811 573 (2012) Available at: http://www-odi.nhtsa.dot.gov/acms/cs/jaxrs/download/doc/UCM399393/INRP-PE11037-49880.pdf (Accessed: 23rd December 2014).
National Transportation Safety Board (NTSB). Auxiliary power unit battery fire Japan Airlines Boeing 787-8, JA829J, Boston, Massachusetts, January 7, 2013, Aircraft Incident Report NTSB/AIR-14/01 PB2014-108867 (2014) Available at: http://www.ntsb.gov/investigations/AccidentReports/Reports/AIR1401.pdf (Accessed: 15th May 2015).
Mikolajczak, C., Kahn, M., White, K. & Long, R. T. Lithium-ion battery failures in Lithium-ion batteries hazard and use assessment, Ch. 4, 43–70, 10.1007/978-1-4614-3486-3_4 (Springer-Verlag, 2011).
Doughty, D. H. & Pesaran, A. A. Vehicle battery safety roadmap guidance, Subcontract Report NREL/SR-5400-54404 (2012) Available at: http://www.nrel.gov/docs/fy13osti/54404.pdf (Accessed: 23rd December 2014).
Wu, H., Zhuo, D., Kong, D. & Cui, Y. Improving battery safety by early detection of internal shorting with a bifunctional separator. Nat. Commun. 5, 5193, 10.1038/ncomms6193 (2014).
Santhanagopalan, S., Ramadass, P. & Zhang, J. Analysis of internal short-circuit in a lithium ion cell. J. Power Sources 194, 550–557, 10.1016/j.jpowsour.2009.05.002 (2009).
Maleki, H. & Howard, J. N. Internal short circuit in Li-ion cells. J. Power Sources 191, 568–574, 10.1016/j.jpowsour.2009.02.070 (2009).
Cai, W., Wang, H., Maleki, H., Howard, J. & Lara-Curzio, E. Experimental simulation of internal short circuit in Li-ion and Li-ion-polymer cells. J. Power Sources 196, 7779–7783, 10.1016/j.jpowsour.2011.04.024 (2011).
Fang, W., Ramadass, P. & Zhang, Z. Study of internal short in a Li-ion cell-II. Numerical investigation using a 3D electrochemical-thermal model. J. Power Sources 248, 1090–1098, 10.1016/j.jpowsour.2013.10.004 (2014).
Zhao, W., Luo, G. & Wang, C. Y. Modeling nail penetration process in large-format li-ion cells. J. Electrochem. Soc. 162, A207–A217, 10.1149/2.1071501jes (2015).
Leising, R. A., Palazzo, M. J., Takeuchi, E. S. & Takeuchi, K. J. Abuse testing of lithium-ion batteries - Characterization of the overcharge reaction of LiCoO2/graphite cells. J. Electrochem. Soc. 148, A838–A844, 10.1149/1.1379740 (2001).
Leising, R. A., Palazzo, M. J., Takeuchi, E. S. & Takeuchi, K. J. A study of the overcharge reaction of lithium-ion batteries. J. Power Sources 97-98, 681–683, 10.1016/S0378-7753(01)00598-5 (2001).
Zeng, Y., Wu, K., Wang, D., Wang, Z. & Chen, L. Overcharge investigation of lithium-ion polymer batteries. J. Power Sources 160, 1302–1307, 10.1016/j.jpowsour.2006.02.009 (2006).
Forgez, C., Vinh, Do, D., Friedrich, G., Morcrette, M. & Delacourt, C. Thermal modeling of a cylindrical LiFePO4/graphite lithium-ion battery. J. Power Sources 195, 2961–2968, 10.1016/j.jpowsour.2009.10.105 (2010).
Li, Z. et al. Examining temporal and spatial variations of internal temperature in large-format laminated battery with embedded thermocouples. J. Power Sources 241, 536–553, 10.1016/j.jpowsour.2013.04.117 (2013).
Zhang, G. S. et al. In situ measurement of radial temperature distributions in cylindrical li-ion cells. J. Electrochem. Soc. 161, A1499–A1507, 10.1149/2.0051410jes (2014).
Rezvanizaniani, S. M., Liu, Z., Chen, Y. & Lee, J. Review and recent advances in battery health monitoring and prognostics technologies for electric vehicle (EV) safety and mobility. J. Power Sources, 256, 110–124, 10.1016/j.jpowsour.2014.01.085 (2014).
Barnett, B., Ofer, D., Sriramulu, S. & Stringfellow, R. Lithium-ion batteries, safety in Batteries for sustainability: selected entries from the encyclopedia of sustainability science and technology (ed. R. J. Brodd ) Ch. 9, 285–318, 10.1007/978-1-4614-5791-6_9 (Springer, 2013).
McCoy, C., Sriramulu, S., Stringfellow, R., Ofer, D. & Barnett, B. Lithium-ion battery safety: detection of developing internal shorts and suppression of thermal runaway. 46thPower Sources Conference, Orlando, Florida, (June 9-12, 2014). Available at: http://www.camxpower.com/wp-content/uploads/4-6.pdf (Accessed: 15th May 2015).
Srinivasan, R. Monitoring dynamic thermal behavior of the carbon anode in a lithium-ion cell using a four-probe technique. J. Power Sources 198, 351–358, 10.1016/j.jpowsour.2011.09.077 (2012).
Schmidt, J. P. et al. Measurement of the internal cell temperature via impedance: Evaluation and application of a new method. J. Power Sources 243, 110–117, 10.1016/j.jpowsour.2013.06.013 (2013).
Sahraei, E., Campbell, J. & Wierzbicki, T. Modeling and short circuit detection of 18650 Li-ion cells under mechanical abuse conditions. J. Power Sources 220, 360–372, 10.1016/j.jpowsour.2012.07.057 (2012).
Wenger, M., Waller, R., Lorentz, V. R. H., Marz, M. & Herold M. Investigation of gas sensing in large lithium-ion battery systems for early fault detection and safety improvement. Industrial Electronics Society, IECON 2014 - 40th Annual Conference of the IEEE, Dallas, TX, 5654 - 5659, doi: 10.1109/IECON.2014.7049366 (October 29 - November 1, 2014).
Campion, C. L., Li, W. & Lucht, B. L. Thermal decomposition of LiPF6-based electrolytes for lithium-ion batteries. J. Electrochem. Soc. 152, A2327–A2334, 10.1149/1.2083267 (2005).
Hammami, A., Raymond, N. & Armand, M. Lithium-ion batteries: Runaway risk of forming toxic compounds. Nature 424, 635–636, 10.1038/424635b (2003).
Arora, P. & Zhang, Z. Battery Separators. Chem. Rev. 104, 4419–4462, 10.1021/cr020738u (2004).
Roth, E. P., Doughty, D. H. & Pile, D. L. Effects of separator breakdown on abuse response of 18650 Li-ion cells. J. Power Sources 174, 579–583, 10.1016/j.jpowsour.2007.06.163 (2007).
Zu, C. X. & Li, H. Thermodynamic analysis on energy densities of batteries. Energy Environ. Sci. 4, 2614–2624, 10.1039/C0EE00777C (2011).
Bruce, P. G., Freunberger, S. A., Hardwick, L. J. & Tarascon, J.-M. Li-O2 and Li-S batteries with high energy storage. Nat. Mater. 11, 19–29, 10.1038/nmat3191 (2012).
Tagawa, K. & Brodd, R. J. Production processes for fabrication of lithium-ion batteries, lithium-ion batteries (eds Masaki, Yoshio, Ralph J, Brodd & Akiya, Kozawa ) Ch. 8, 181–194, 10.1007/978-0-387-34445-4_8 (Springer, 2009).
Acknowledgements
The authors gratefully acknowledge funding from The United States Department of Energy (CAEBAT Program) through a subcontract from EC Power. G.Z. also thanks Kamiar Salehi of ECEC for assistance in building the experimental system and Dr. Bangzhi Liu from Materials Research Institute for help in parylene coating of micro sensors. The work of parylene coating was partially supported by the Pennsylvania State University Materials Research Institute Nanofabrication Lab and the National Science Foundation Cooperative Agreement No. ECS-0335765.
Author information
Authors and Affiliations
Contributions
C.W. and G.Z. conceived the idea. L.C., S.G. and G.Z. fabricated experimental cells. G.Z. performed tests. G.Z., C.W., C.S. and C.R. discussed about the results. G.Z. and C.W. co-wrote the manuscript. All authors made comments on the manuscript.
Ethics declarations
Competing interests
The authors declare no competing financial interests.
Electronic supplementary material
Rights and permissions
This work is licensed under a Creative Commons Attribution 4.0 International License. The images or other third party material in this article are included in the article’s Creative Commons license, unless indicated otherwise in the credit line; if the material is not included under the Creative Commons license, users will need to obtain permission from the license holder to reproduce the material. To view a copy of this license, visit http://creativecommons.org/licenses/by/4.0/
About this article
Cite this article
Zhang, G., Cao, L., Ge, S. et al. Reaction temperature sensing (RTS)-based control for Li-ion battery safety. Sci Rep 5, 18237 (2015). https://doi.org/10.1038/srep18237
Received:
Accepted:
Published:
DOI: https://doi.org/10.1038/srep18237
This article is cited by
-
Lithium-ion Battery Thermal Safety by Early Internal Detection, Prediction and Prevention
Scientific Reports (2019)
-
Mechanism of the entire overdischarge process and overdischarge-induced internal short circuit in lithium-ion batteries
Scientific Reports (2016)
Comments
By submitting a comment you agree to abide by our Terms and Community Guidelines. If you find something abusive or that does not comply with our terms or guidelines please flag it as inappropriate.